Sponsored Content by MerckJan 22 2024Reviewed by Emily Magee
Fibrous materials are playing an increasingly vital role in various applications, including textiles, nonwoven materials, composite structures, and more.
Polymeric nanofibers represent an exciting new material category, gaining substantial attention due to their exceptional characteristics, including a high specific surface area, pronounced porosity, strong molecular alignment, and nanoscale effects (Figure 1A).1
Nanofibers also facilitate the convenient inclusion of various therapeutic molecules, enhancing drug loading capacity and supporting sustained release (Figure 1B). Moreover, manipulating polymeric fibers on a macroscopic scale opens up potential applications as implantable local drug delivery platforms.

Figure 1. A) Schematic images of film and nonwoven nanofiber meshes. B) Examples of the different types of molecules that can be easily incorporated into nanofibers. Image Credit: Merck
Nanofibers can be produced using various methods, including phase separation, self-assembly, electrospinning, drawing, and microfluidic techniques.2
Electrospinning, widely researched, provides a cost-effective and straightforward means of producing continuous fibers with uniform diameters ranging from micro- to nanometers.3 Its versatility allows the processing of nearly any soluble or fusible polymer into nanofibers.
Recently, the scientific community has rekindled interest in expanding the repertoire of functional nanofibers for diverse applications. For instance, nanofibers with varied compositions can be prepared by electrospinning polymers blended with nanoparticles, carbon nanotubes, ceramics, biomolecules, and other substances.4
These functional nanofibers exhibit potential in applications such as tissue engineering, sensors, and wound healing. Additionally, nanofibers with "smart" properties have piqued interest for applications involving "on-off" switchable control of permeability, wettability, and swelling/deswelling behavior.5
Certain polymers find application in creating intelligent nanofibers. These polymers receive various names based on their physical or chemical attributes, such as "stimuli-responsive," "environmentally sensitive," "smart," or "intelligent" polymers. To maintain consistency and clarity, the term "smart polymers" will be consistently employed throughout the remaining sections of this article.
One defining characteristic that underscores the intelligence of nanofibers is their capacity to react to subtle changes in the surrounding environment. The rapid macroscopic alterations in their structure and the reversible nature of these transitions underscore the distinctive qualities of these materials.
Smart polymers' nanofibers exhibit swift response rates owing to their inherent design features.6 This article concentrates on the applications of smart nanofiber meshes as a localized implantable drug delivery platform.
It introduces four types of nanofibers, as depicted in Figure 2. These encompass nanofibers with sustained drug release properties (Type A), on-off drug release properties (Type B), and a combination of one of these types with thermo-therapy (referred to as Type C and D, respectively), made feasible by the incorporation of a heat source.
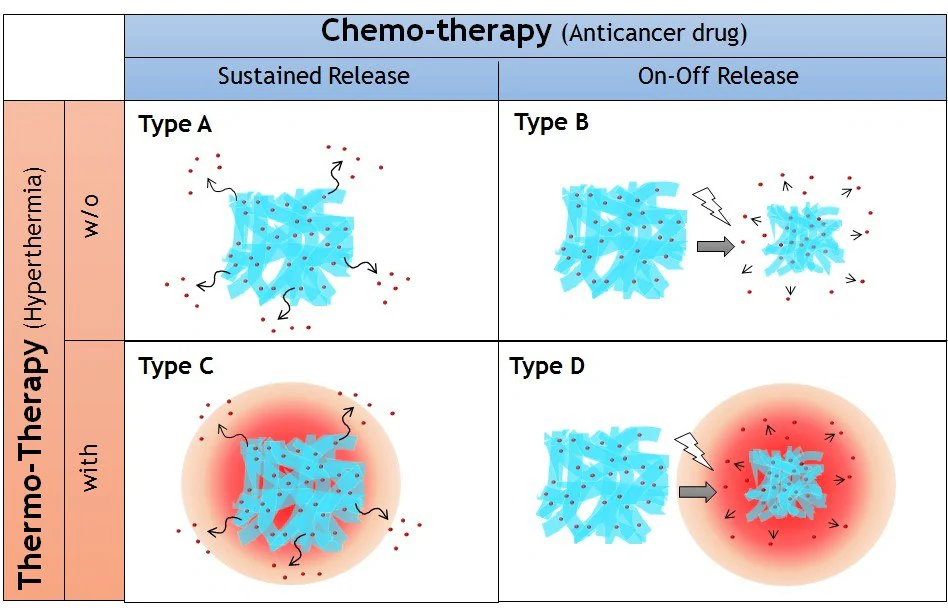
Figure 2. Four types of implantable nanofiber system for local drug delivery system proposed in this study. Nanofibers with sustained (Type A) and on-off (Type B) drug release properties combined with thermotherapy (Type C and D, respectively). Image Credit: Merck
Drug release control
Sustained release (Type A)
An example of intelligent material employing controlled release technology is a nanofiber lattice designed for peripheral nerve injuries. When a wound induces harm to peripheral nerves, the patient may experience motor and sensory paralysis, along with autonomic nerve dysfunction.
Typical approaches to address peripheral nerve injuries involve directly stitching the cut or procuring and transplanting nerves from another part of the body. Recently, strides have been made in the advancement of synthetic nerves employed to facilitate nerve restoration.
A nanofiber mesh that directly encases the impaired peripheral nerves has been developed, fostering regeneration and reinstating functionality.
The nanofiber lattice is crafted from poly(e-caprolactone) (PCL), a biodegradable aliphatic polyester sanctioned by the US Food and Drug Administration (FDA) for biomedical applications such as delivering drugs, scaffolding for tissue engineering, and materials for implants.7
Researchers synthesized PCL by ring-opening polymerization from terminal hydroxyl groups of an initiator using tin octoate (S3252) as a catalyst.8 PCL nanofibers were generated through an electrospinning method using 1,1,1,3,3,3-hexafluoroisopropanol (HFIP, 105228) as a solvent.
The mesh incorporates methylcobalamin (MeCbl), an active vitamin B12 analog, efficiently delivered to nerve tissues, effectively stimulating nerve regeneration and preserving neuronal cell viability. PCL nanofiber meshes gradually dispensed MeCbl over a minimum of 8 weeks of in vitro tests (Figure 3A).
Localized implantation of nanofiber sheets with effectively incorporated MeCbl contributed to the restoration of motor and sensory function, improved nerve conduction velocity, and encouraged myelination after sciatic nerve injury without impacting MeCbl plasma concentration.9
The team also examined the PCL nanofiber systems with an immune-modulating agent (Figure 3B),10 inactivated virus (Figure 3C),11 and microRNA (Figure 3D) for the treatment of basal cell carcinoma, prostate cancer cells, and liver cancer cells, respectively.
These instances underscore the benefits of nanofiber systems in which various molecules can be effortlessly integrated, irrespective of molecular size, shape, surface charge, and hydrophilicity.
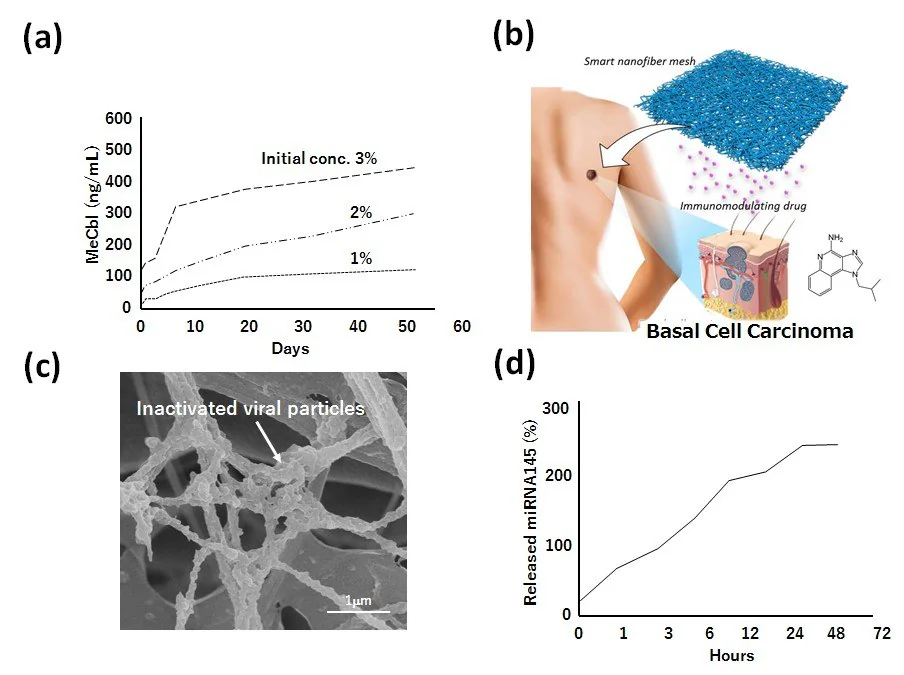
Figure 3. A) Drug release behavior of methylcobalamin (MeCbl) from PCL nanofiber meshes. B) Schematic image of immunomodulating drug-incorporated nanofiber mesh for basal cell carcinoma therapy. C) Scanning electron microscopic image of inactivated viral particlesmodified PCL nanofiber mesh for prostate cancer therapy. D) Drug release behavior of micro RNA 145 from PCL nanofiber mesh. Image Credit: Merck
On-off switchable release (Type B)
In addition to maintaining a steady release, precise timing of drug administration plays a crucial role in effective chemotherapy. A recent approach known as "dose-dense chemotherapy" has gained attention for its focus on maximizing tumor destruction by accelerating the rate of chemotherapy delivery rather than increasing the dosage.
Administering chemotherapy weekly, as opposed to every three weeks, enables the treatment to disrupt the rapid growth phase of tumor cells. In this context, intelligent nanofiber meshes have the potential to facilitate temporal drug release triggered by external stimuli.
A significant challenge in developing these smart nanofiber meshes lies in designing nanofibers with dynamically and reversibly adjustable structures on both the nano- and macroscopic scales.
This was accomplished by using electrospun copolymers of N-isopropylacrylamide (NIPAAm, 731129) with a UV-reactive benzophenone (BP) conjugated co-monomer.14
These photo-crosslinkable, temperature-responsive polymer-based nanofiber meshes exhibited adjustable properties, including swelling/shrinking, mechanical strength, and porosity. A drawback of this method is that the copolymer's phase transition temperature decreases after conjugation due to the hydrophobic nature of BP.
Consequently, smart nanofibers were also created by electrospinning copolymers of NIPAAm and N-hydroxymethyl acrylamide (HMAAm) because the hydroxyl groups of HMAAm are subsequently cross-linked by thermal curing.
The successful transformation of copolymers into a well-defined nanofibrous structure with a diameter of approximately 600–700 nm was achieved, maintaining the fibers' morphology even after thermal curing.
The resulting cross-linked nanofibers displayed rapid and reversible volume changes in aqueous media in response to cycles of temperature alternation around body temperature.15 The on-off switchable release of dextran from the cross-linked nanofibers was observed.
Nearly all the dextran was released from the nanofibers after six heating cycles, with only a negligible amount released during the cooling process. These drug release patterns demonstrate that the proposed system can release a specific amount of a drug in accordance with the circadian rhythm of the disease.
This feature makes the system suitable for the optimal treatment of chronic diseases exhibiting circadian variation, such as asthma, hypertension, myocardial infarction, and arthritis.
Combination with hyperthermia
Sustained release with heat (Type C)
Although chemotherapy has historically stood as the standard treatment for primary and metastatic cancer, its clinical benefits are still limited when administering a single anticancer drug.
As a result, clinical practitioners have turned to combination chemotherapy regimens involving two or more traditional anticancer drugs to address various cancers in recent decades.
Numerous clinical trials have demonstrated a noteworthy decrease in tumor size when coupling chemotherapy with additional treatments like radiotherapy and thermal therapy.16 Thermal therapy, or hyperthermia, involves subjecting body tissues to elevated temperatures (43–45 °C).
Hyperthermia can also amplify the efficacy of specific anticancer drugs, such as paclitaxel (PTX), heightening the sensitivity of certain cancer cells to treatment.
Taking these aspects into account, a smart nanofiber system has been devised, integrating chemotherapy and hyperthermia for a more potent cancer therapy (Figure 4A).17 The mesh comprises biodegradable PCL with PTX and magnetic nanoparticles (MNPs) (Figure 4B).
In vitro testing revealed that the PCL mesh gradually released PTX for a minimum of 6 weeks. In vivo, this extended therapeutic effect manifested as a continuous release of medication from the mesh over an extended period, contrasting with the direct injection of PTX into the tumor site.
Additionally, the mesh, when stimulated with an alternating magnetic field (AMF), demonstrated a synergistic anticancer effect. This occurred because the MNPs within the nanofiber generated localized heat, resulting in heat-induced cell death and an augmented chemotherapeutic impact of PTX.
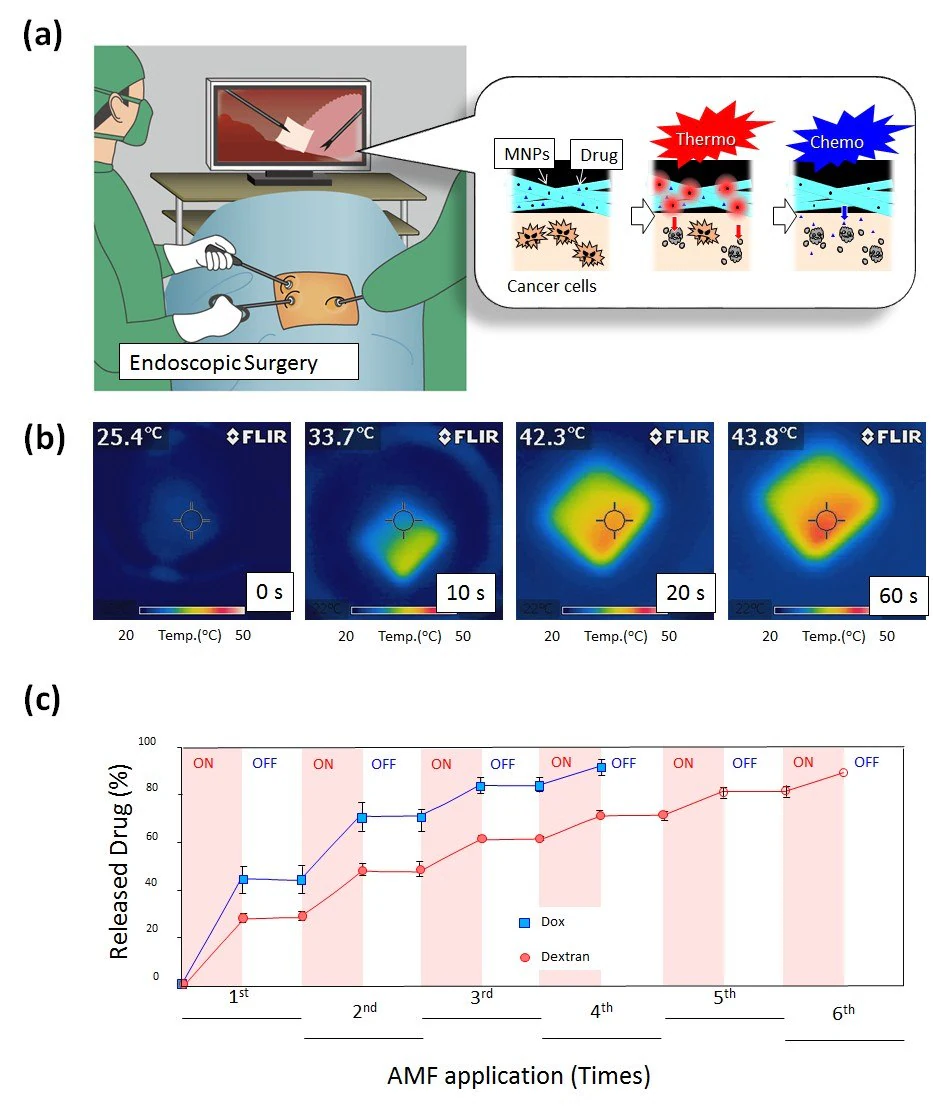
Figure 4. A) Schematic illustration for on-off switchable temperature-responsive fiber mesh for cancer thermo-chemotherapy. B) The infrared thermal images of MNPs-loaded nanofiber mesh in AMF. C) On-off switchable drug release profiles for DOX and dextran from temperature-responsive nanofiber meshes in response to AMF application. Image Credit: Merck
Building upon these findings, the smart nanofiber system exhibits potential for future cancer therapeutics and may offer fresh insights into the forthcoming development of localized thermo/chemotherapy.
On-off switchable release with heat (Type D)
Drug delivery systems that can be switched on and off have garnered attention in relation to circadian clocks.18 Programmable drug delivery systems, synchronized with specific times, are utilized in chronotherapeutics.
Combining this approach with hyperthermia treatments enhances PTX-induced apoptosis by activating caspase-7, increasing G2/M cell arrest, and consequently reducing PTX's IC50.
Against this backdrop, a smart hyperthermia nanofiber system has been under development, featuring simultaneous heat generation and drug release in response to the on-off switching of alternating magnetic fields (AMF) to induce cancer apoptosis.19
The nanofiber comprises a chemically crosslinkable temperature-responsive polymer, poly(NIPAAm-co-HMAAm), incorporating an anticancer drug (doxorubicin; DOX) and magnetic nanoparticles (MNPs). The mesh is fabricated using an electrospinning method.
Through chemical crosslinking, the nanofiber mesh exhibits switchable changes in swelling ratio in response to alternating on-off switches of AMF.
The self-generated heat from the embedded MNPs induces deswelling of polymer networks in the nanofiber, enabling the corresponding on-off release of DOX from the nanofibers in response to AMF (Figure 4C).
Approximately 70% of human melanoma cells succumbed to a 5-minute application of AMF in the presence of MNPs and DOX-loaded nanofibers, benefiting from the dual effect of heat and drug.
This smart hyperthermia approach has also been applied to lung adenocarcinoma, with the phase transition temperature of the temperature-responsive mesh adjusted to the mild hyperthermia temperature range of around 43 °C.
In vitro anti-tumor studies revealed that both MNP- and PTX-loaded meshes killed about 66% of cells, while meshes loaded only with PTX killed approximately 43% of cells.20
In a mouse lung cancer model, the thermo-chemotherapy combination exhibited enhanced anti-tumor activity, eliminating systemic toxic effects on the mice due to the local release of chemotherapeutic agents.
It is believed that such fiber systems could serve as a model to inform the design of the next generation of local drug delivery systems, ensuring safe and effective cancer treatment.
Conclusions
Various types of fibers at different production stages have been explored for numerous applications across diverse fields, such as nanotechnology, textiles, industry, fuel cells, tissue engineering, regenerative medicine, and biomaterials.
Notably, well-defined nanofibers exhibit remarkable features in comparison to other material types, including high specific surface area, significant porosity, and biomimetic properties, offering promising potential applications.
Within this array of fibers, those capable of integration with stimuli-responsive media are referred to as smart fibers. These smart fibers can be remotely controlled through external stimuli, preserving their original characteristics.
The dynamic nature of smart fibers also enables the generation of mechanical forces, influencing changes in cellular behavior.21 Considering the merits of smart fibers, these materials can serve as versatile tools across a broad spectrum of applications.
Acknowledgments
The author expresses gratitude for the financial support provided by JSPS KAKENHI (Grant Number 264086 and 26750152). Additionally, the author acknowledges Prof. Allan S. Hoffman (University of Washington) for ongoing and valuable commentary and discussions.
References
- Niiyama E, Niiyama E, Fulati A, Fulati A, Ebara M, Ebara M, Ebara M. 2018. Responsive Polymers for Smart Textiles.111-126. https://doi.org/10.1002/9781119460367.ch4
- Ebara M, Kotsuchibashi Y, Narain R, Idota N, Kim Y, Hoffman JM, Uto K, Aoyagi T. 2014. Smart Biomaterials. https://doi.org/10.1007/978-4-431-54400-5
- Namekawa K, Tokoro Schreiber M, Aoyagi T, Ebara M. 2014. Fabrication of zeolite?polymer composite nanofibers for removal of uremic toxins from kidney failure patients. Biomater. Sci.. 2(5):674. https://doi.org/10.1039/c3bm60263j
- Takai R, Kurimoto R, Nakagawa Y, Kotsuchibashi Y, Namekawa K, Ebara M. 2016. Towards a Rational Design of Zeolite-Polymer Composite Nanofibers for Efficient Adsorption of Creatinine. Journal of Nanomaterials. 20161-7. https://doi.org/10.1155/2016/5638905
- Bou S, Ellis AV, Ebara M. 2016. Synthetic stimuli-responsive ?smart? fibers. Current Opinion in Biotechnology. 39113-119. https://doi.org/10.1016/j.copbio.2016.03.009
- Maeda T, Kim Y, Aoyagi T, Ebara M. The Design of Temperature-Responsive Nanofiber Meshes for Cell Storage Applications. Fibers. 5(1):13. https://doi.org/10.3390/fib5010013
- Uto K, Muroya T, Okamoto M, Tanaka H, Murase T, Ebara M, Aoyagi T. 2012. Design of super-elastic biodegradable scaffolds with longitudinally oriented microchannels and optimization of the channel size for Schwann cell migration. Science and Technology of Advanced Materials. 13(6):064207. https://doi.org/10.1088/1468-6996/13/6/064207
- Ebara M, Uto K, Idota N, Hoffman JM, Aoyagi T. 2012. Shape-Memory Surface with Dynamically Tunable Nano-Geometry Activated by Body Heat. Adv. Mater.. 24(2):273-278. https://doi.org/10.1002/adma.201102181
- Suzuki K, Tanaka H, Ebara M, Uto K, Matsuoka H, Nishimoto S, Okada K, Murase T, Yoshikawa H. 2017. Electrospun nanofiber sheets incorporating methylcobalamin promote nerve regeneration and functional recovery in a rat sciatic nerve crush injury model. Acta Biomaterialia. 53250-259. https://doi.org/10.1016/j.actbio.2017.02.004
- Garrett R, Niiyama E, Kotsuchibashi Y, Uto K, Ebara M. Biodegradable Nanofiber for Delivery of Immunomodulating Agent in the Treatment of Basal Cell Carcinoma. Fibers. 3(4):478-490. https://doi.org/10.3390/fib3040478
- Okada T, Uto K, Aoyagi T, Ebara M. A biomimetic approach to hormone resistant prostate cancer cell isolation using inactivated Sendai virus (HVJ-E). Biomater. Sci.. 4(1):96-103. https://doi.org/10.1039/c5bm00375j
- Che H, Lee HJ, Uto K, Ebara M, Kim WJ, Aoyagi T, Park I. 2015. Simultaneous Drug and Gene Delivery from the Biodegradable Poly(?-caprolactone) Nanofibers for the Treatment of Liver Cancer. j nanosci nanotechnol. 15(10):7971-7975. https://doi.org/10.1166/jnn.2015.11233
- Katsumata N. 2013. Dose-dense effect: other contributors ? Author's reply. The Lancet Oncology. 14(12):e489-e490. https://doi.org/10.1016/s1470-2045(13)70488-1
- 14. Kim Y, Ebara M, Aoyagi T. 2013. A Smart Hyperthermia Nanofiber with Switchable Drug Release for Inducing Cancer Apoptosis. Adv. Funct. Mater.. 23(46):5753-5761. https://doi.org/10.1002/adfm.201300746
- Kim Y, Ebara M, Aoyagi T. 2012. Temperature-responsive electrospun nanofibers for ?on?off? switchable release of dextran. Science and Technology of Advanced Materials. 13(6):064203. https://doi.org/10.1088/1468-6996/13/6/064203
- URANO M. 1999. Invited Review: For the clinical application of thermochemotherapy given at mild temperatures. International Journal of Hyperthermia. 15(2):79-107. https://doi.org/10.1080/026567399285765
- Niiyama E, Ebara M. 2019. Smart Nanofiber Meshes for Cancer Thermo/Chemotherapy. Official Journal of the Japan Society of Drug Delivery System. 34(3):179-185. https://doi.org/10.2745/dds.34.17
- Lévi F, Okyar A. 2011. Circadian clocks and drug delivery systems: impact and opportunities in chronotherapeutics. Expert Opinion on Drug Delivery. 8(12):1535-1541. https://doi.org/10.1517/17425247.2011.618184
- Kim Y, Ebara M, Aoyagi T. 2012. A Smart Nanofiber Web That Captures and Releases Cells. Angew. Chem. Int. Ed.. 51(42):10537-10541. https://doi.org/10.1002/anie.201204139
- Niiyama E, Uto K, Lee C, Sakura K, Ebara M. Alternating Magnetic Field-Triggered Switchable Nanofiber Mesh for Cancer Thermo-Chemotherapy. Polymers. 10(9):1018. https://doi.org/10.3390/polym10091018
- Mano SS, Uto K, Ebara M. 2017. Material-induced Senescence (MIS): Fluidity Induces Senescent Type Cell Death of Lung Cancer Cells via Insulin-Like Growth Factor Binding Protein 5. Theranostics. 7(19):4658-4670. https://doi.org/10.7150/thno.20582
About Merck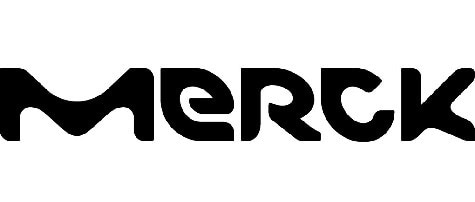
Our pursuit is progress for people everywhere. That's why we take a closer look at things, ask questions, and think ahead.
We've been around for more than 350 years, yet our majority owners are still the descendants of Friedrich Jacob Merck, the man who founded our company in Darmstadt, Germany in 1668.
From advancing gene-editing technologies and discovering unique ways to treat the most challenging diseases to enabling the intelligence of devices – the company is everywhere.
We are Merck. The only exceptions are the United States and Canada. Here we operate as EMD Serono in the Biopharma business, as MilliporeSigma in the Life Science business, and as EMD Performance Materials in the materials business.
Our life science business
We provide infinite solutions to solve the toughest problems in life science in collaboration with the global scientific community. Our tools, services, and digital platforms empower scientists and engineers at every stage, helping deliver breakthrough therapies more quickly.
Focus areas
With our three business units, we are a leading worldwide supplier of tools, high-grade chemicals, and equipment for academic labs, biotech, and biopharmaceutical manufacturers, as well as the industrial sector.
- Research Solutions provides our academic customers with the chemicals and tools needed to make scientific discovery easier and faster.
- Process Solutions provides drug manufacturers with process development expertise and technologies, such as continuous bioprocessing.
- Applied Solutions offers both testing kits and services to ensure that our food is safe to eat and our water is clean to drink.
Sponsored Content Policy: News-Medical.net publishes articles and related content that may be derived from sources where we have existing commercial relationships, provided such content adds value to the core editorial ethos of News-Medical.Net which is to educate and inform site visitors interested in medical research, science, medical devices and treatments.