Sponsored Content by MerckJan 22 2024Reviewed by Emily Magee
The Flash NanoPrecipitation (FNP) concept, initially presented in 2003 by Johnson and Prud’homme, introduces an innovative approach to creating highly concentrated nanoparticles through a swift mixing and precipitation procedure.1
Image Credit: David A Litman/Shutterstock.com
FNP technology has evolved, influencing diverse fields, from drug encapsulation to pesticide delivery. It employs a scalable mixing method capitalizing on the distinct timescales of nucleation, aggregation, and stabilization.
In its simplest form, a profoundly hydrophobic molecule and an amphiphilic polymer dissolve in a water-miscible organic solvent. This organic stream is then mixed rapidly with an aqueous anti-solvent stream using specially designed turbulent mixing chambers. During this process, concurrent kinetic activities unfold:
- Nucleation and aggregation of the hydrophobic active;
- Adsorption of the steric stabilizing polymer on the aggregate, ultimately impeding further growth.
The ratio of active-to-polymer controls size; with insufficient polymer, nanoparticles grow too large, and with excess stabilizing polymer, micelles may form.
Beyond a certain mixing intensity threshold (Reynolds number, Re), where mixing time surpasses nanoparticle growth time, nanoparticle size becomes independent of flow rate, relying solely on stream composition.2
Turbulence, a scalable factor, is crucial to this mixing and differs fundamentally from microfluidic device flows, inherently involving low Reynolds numbers.
Evolution of mixers and scalability
The original mixer design involved two opposing streams of equal momentum colliding in a cylindrical mixing volume. Utilizing handheld syringes or syringe pumps to propel the streams, this confined impinging jet (CIJ) mixer facilitated small-batch production for swift screening and formulation development (Figure 1).
The stability of nanoparticle size post-formation is influenced by the rate of growth through Ostwald ripening, a process that hinges on the solubility of the active ingredient in the solution phase.3 As the CIJ mixer demands equal stream velocities, the resulting solvent:antisolvent ratio remains 1:1.
To decrease the antisolvent ratio and active solubility, the CIJ output can be collected into a quench bath, typically resulting in a final solvent concentration of 10%.
Liu et al. introduced a novel mixer design employing four mixing streams with tangential turbulent flow (Figure 1). Dubbed the multi-inlet vortex mixer (MIVM), each MIVM stream independently contributes to the micro-mixing process.4
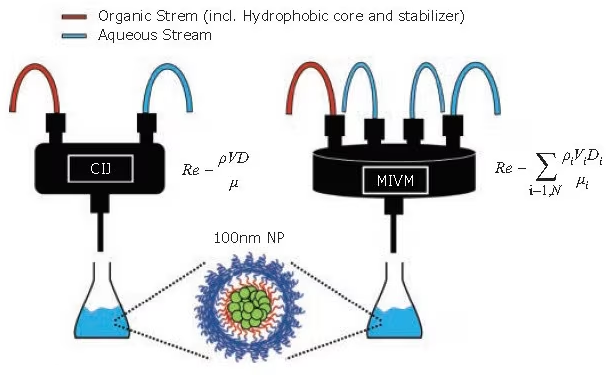
Figure 1. Schematic of the FNP process at various scales. (Left) The CIJ mixer allows for the synthesis of many small batches in rapid succession for screening formulations. (Right) The MIVM can scale up formulations to produce liters of nanoparticle solutions. (Equations for Reynolds number, Re, defined in refs. 1 and 4). Image Credit: Merck
In contrast to the CIJ mixer, the MIVM does not need to match the momenta of the streams, providing more flexibility in nanoparticle formulations. For instance, two distinct organic solvents can be used in separate streams, each carrying a different API.
If adequate dilution of the organic takes place in the mixing step, no additional quench bath is needed, enabling a genuinely continuous nanoparticle production process.
Markwalter et al. expanded the MIVM design by developing a downsized version that demands less material per batch of nanoparticles.5 A side-by-side comparison of mixing devices of varying scales revealed no significant variation in particle size or properties as long as sufficient mixing intensity is maintained.6
Comparisons between the MIVM and CIJ mixers indicate identical nanoparticle sizes and distributions beyond a threshold Re number (Figure 2B). Dynamic light scattering (DLS) traces and TEM images of nanoparticles formulated using the MIVM and CIJ also exhibit no visible differences in size or properties (Figure 2A, 2C, 2D).6
This facilitates a seamless development process where screening and small-scale formulations are conducted using the CIJ mixer and then scaled up using the MIVM. A large-scale MIVM is currently in use at WuXi AppTec (Shanghai) to produce 250 nm nanoparticles of an antimalarial drug at 2 L/min in 300 L batches.
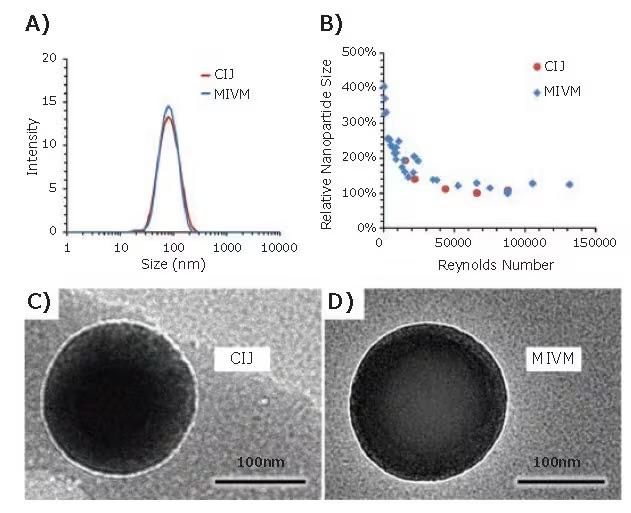
Figure 2. Comparison of nanoparticle formulations by CIJ and MIVM. (A) DLS trace of nanoparticles formulated with Vitamin E acetate core and PS-b-PEG stabilizer. Nanoparticle size is independent of the mixer type. (B) Relative nanoparticle size vs. mixing Reynolds number for CIJ and MIVM formulations. Above a threshold Reynolds number of 50000, nanoparticle size is insensitive to flow rate. (C, D) TEM images of CIJ and MIVM nanoparticles show no significant differences between nanoparticles made by the two mixer types. Image Credit: Merck
Stabilizer selection
As mentioned earlier, the amphiphilic stabilizer plays a crucial role in nanoparticle formation. For the majority of FNP applications, initial screening typically involves non-degradable block copolymers. Polystyrene-block-polyethylene glycol (PS-b-PEG) serves as a versatile stabilizer for many applications.
When integrated into a nanoparticle, the hydrophilic PEG chains form a dense outer corona around the hydrophobic core. This dense PEG layer imparts favorable biological properties such as extended circulation times and mucus penetration.7
Substituting some or all of the PEG copolymer with polyacrylic acid (PAA) or 2-(dimethylamino) ethyl methacrylate (DMEMA) can give the nanoparticle negative or positive surface charges, respectively.
Since surface charge influences nanoparticle biodistribution and clearance, adjusting the surface charge can aid in targeting nanoparticles to specific tissues of interest. For applications requiring biodegradable nanoparticles, the polystyrene block can be replaced with either polylactic acid (PLA) or polycaprolactone (PCL).8
The hydrolytic breakdown of these polymers enables a gradual and consistent release of drugs from the nanoparticles, leading to eventual clearance. Figure 3 illustrates the chemical structures of commonly utilized block copolymer stabilizers.
In the context of global health applications, production costs dictate the use of lower-cost materials during the formulation process. In such systems, cost-effective stabilizers, such as hydroxypropyl methylcellulose acetate succinate (HPMC-AS), lecithin, zein, or casein, can be employed.9
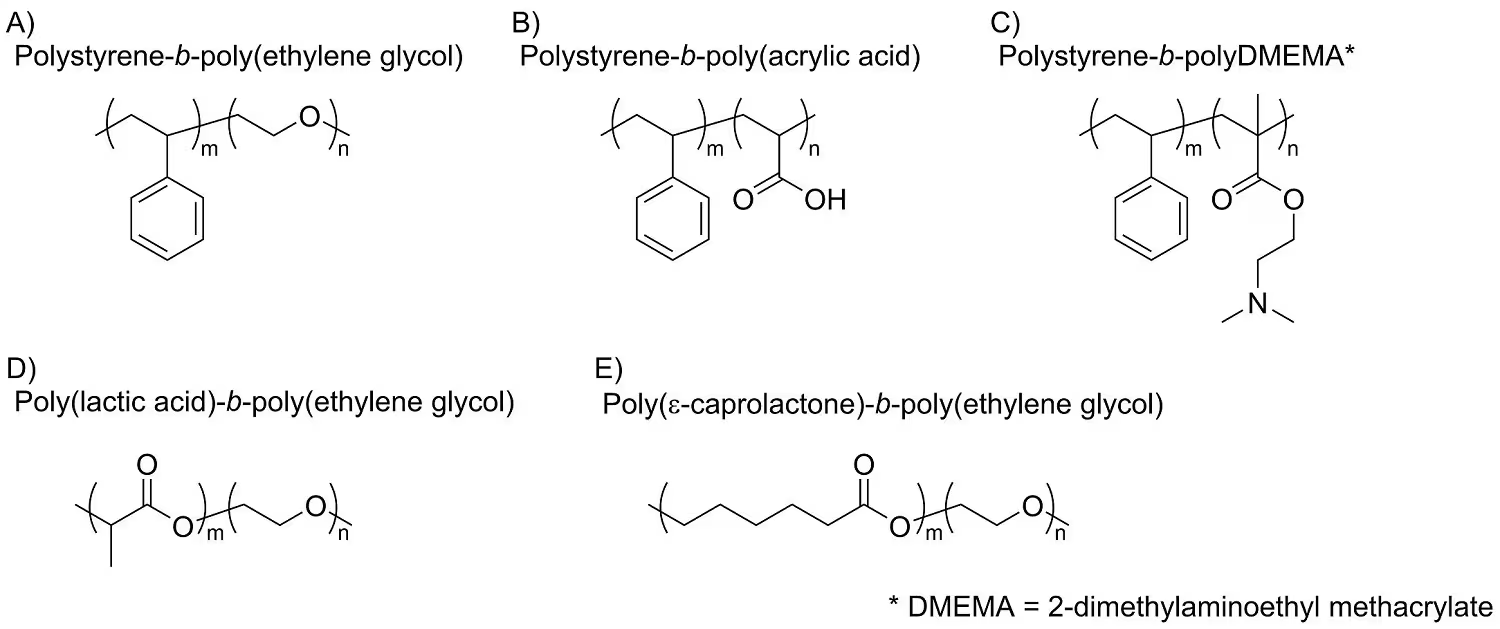
Figure 3. Typical polymeric stabilizers used for FNP. The relatively inexpensive polystyrene-based block co-polymers (A, B, C) are generally used for prototyping or ex-vivo applications. Biodegradable co-polymers (D, E) are used in therapeutic applications. Image Credit: Merck
Applications in oral delivery formulations
One use of FNP involves encapsulating hydrophobic drugs to enhance the bioavailability of the active pharmaceutical ingredient (API). Clofazimine (CFZ), a hydrophobic molecule initially designed for leprosy treatment, has recently shown effectiveness against Cryptosporidium infections, a leading cause of diarrhea in the developing world.
Pure CFZ, however, faces challenges with slow dissolution rates in the gastrointestinal (GI) tract, rendering it unsuitable for the rapid treatment needed for Cryptosporidium. Flash nanoprecipitation enhances solubility and accelerates CFZ dissolution.
Through the fast precipitation and stabilization process of FNP, the hydrophobic active molecule can be retained and preserved in an amorphous state. Additionally, the small size of FNP nanoparticles augments the specific surface area, thereby increasing the drug dissolution rate. Both factors enhance the solubility and oral bioavailability of CFZ.
In the research conducted by Zhang et al., CFZ was encapsulated into nanoparticles with very high encapsulation efficiencies (>92%) using various low-cost stabilizers.9
DSC traces verified that encapsulation into nanoparticles maintained the amorphous state of CFZ (Figure 4A), even after processing the nanoparticles into dry powder through spray drying or lyophilization.
After exposure to simulated gastric and intestinal fluids, CFZ nanoparticles exhibited 50–90x higher supersaturation levels and complete dissolution after 6 hours.
Feng et al. also demonstrated that FNP’s ability to stabilize an API’s amorphous form and enhance dissolution was not exclusive to CFZ.10 Lumefantrine (LMN), another hydrophobic API effective against malaria, experiences poor bioavailability and absorption in the GI tract. In this study, LMN was encapsulated into nanoparticles using low-cost natural polymers.
Similar to the earlier study with CFZ, FNP showed the capability to inhibit the crystallization of LMN and improve dissolution in a simulated intestinal fluid by over two orders of magnitude (Figure 4B).
The amorphous nanoparticle form also reduced the disparity in dissolution between fed-state and fasted-state intestinal fluids. Nanoparticle formulation can decrease the susceptibility of release to environmental conditions, including high temperature or humidity, compared to traditional spray-dried dispersions of the same API.11
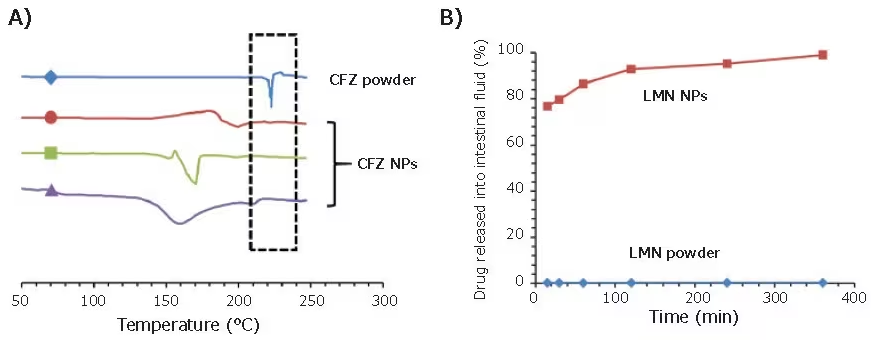
Figure 4. Applications of FNP in the encapsulation of oral therapeutics. (A) DSC trace of three clofazimine nanoparticle formulations. CFZ powder exhibits a crystallization peak at 22 °C, while nanoparticle formulations can preserve CFZ in its amorphous state. (B) Drug release assays of LMN formulations in simulated intestinal fluid. Nanoparticles exhibit close to full release after 2 hours, while LMN powder exhibits <1% drug in solution. Image Credit: Merck
Applications in medical imaging
Contrast agents are commonly utilized in medical diagnostics to facilitate more precise and responsive imaging.
Widely employed contrast agents, like FDG for PET, are directly administered to the patient and, as a result, must possess water solubility. FNP facilitates the encapsulation of water-insoluble compounds, paving the way for a range of previously unavailable hydrophobic contrast agents.
The FNP process excels at producing highly concentrated nanoparticles, which can significantly enhance imaging sensitivity by concentrating contrast agents in a nanoparticle core. For instance, phthalocyanine-based dyes are enclosed at a 35% core loading using PS-b-PEG stabilizer.12
At these elevated core concentrations, nanoparticles can be applied for contrast-assisted photoacoustic imaging. Lu et al. employed photoacoustic imaging to examine the biodistribution of nanoparticles in a mouse tumor model.13
The distinct and well-defined absorbance spectra of the organic dye enable multiplexed imaging, where signals are gathered at different wavelengths and then separated to create a unified image.
With this technology, both nanoparticle and oxygenated/deoxygenated blood signals can be visualized simultaneously (Figure 5A). More conventional fluorescence imaging can also be employed to monitor biodistribution, as depicted in Figure 5B.
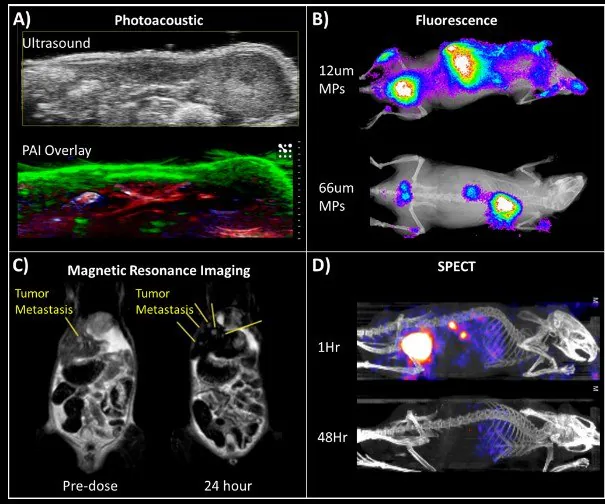
Figure 5. Biological imaging applications of FNP nanoparticles. (A) Multiplex imaging using photoacoustic tomography of a mouse model shows nanoparticle accumulation in the skin (green). Oxygenated and deoxygenated blood can also be visualized (red and blue, respectively). (B) Fluorescence of dye-encapsulated nanoparticles. Biodegradable microparticles containing these nanoparticles were injected into mice. Smaller particles accumulated in the liver while larger particles tracked to the lungs. (C) MRI imaging of liver metastases in a mouse tumor model using iron oxide-based nanocrystals (IONC) encapsulated nanoparticles. Yellow lines point to the locations of liver metastases before and after injection of IONC nanoparticles. Nanoparticles persist in healthy tissues (appearing black) — generating contrast that allows better visualization of metastasis. (D) SPECT imaging of 111In labeled nanoparticles shows biodistribution and clearance profile over time. Image Credit: Merck
In the above depiction of gel microparticles containing fluorescent nanoparticles, the size of microparticles determines their capture in the lungs. Pinkerton et al. broadened the diagnostic applications of FNP to MRI through the encapsulation of iron oxide-based nanocrystals (IONC).14
As a strong T2 MRI signal is highly dependent on IONC size and density, small clusters of IONCs were formulated into nanoparticles at up to 25% wt., using FNP in a single-step assembly process.
Hydroxy-terminated PS-b-PEG was utilized as the stabilizer to boost uptake by liver macrophages. In an NSCLC orthotopic mouse model, these IONCs nanoparticles were capable of enhancing MRI contrast of the liver and facilitating the detection of otherwise imperceptible tumor metastases (Figure 5C).
The use of radiotracer-based PET or SPECT imaging can greatly enhance sensitivity and imaging resolution.15 To achieve this, it is essential to synthesize nanoparticles that can quickly and efficiently “load” these tracers. One method involves attaching a chelating molecule to the hydrophilic end of the stabilizer.
The chelators then capture radiotracers, forming SPECT/PET-active nanoparticles. In a SPECT study, PS-b-PEG conjugated with DPTA (diethylenetriaminepentaacetic acid) was employed to chelate 111In.
These nanoparticles were subsequently utilized in a pharmacokinetic study to measure the clearance mechanism and rate of nanoparticle clearance (Figure 5D). Lu et al. recently presented a technique for radiolabeling PEG-coated nanoparticles with 64-Cu for PET, demonstrating advantages over surface conjugation.
A phthalocyanine-based metal chelator is initially encapsulated, with the hydrophobic phthalocyanine in the nanoparticle core. The nanoparticles then undergo “PET activation” as Cu-64 is loaded into the core through a swift incubation step.16 This core-radiolabeling minimizes potential off-target effects from exposed chelators on the nanoparticle surface.
Applications in targeting specific cell types
Enhancing the precision of nanoparticle delivery to specific organs or cell types can boost the efficacy of treatments and the accuracy of diagnostic imaging. This is accomplished by affixing targeting ligands to the exterior of nanocarriers.
These ligands encompass antibodies, peptides, sugars, and small molecules that selectively bind to receptors on the intended cells. In one example, mannoside-conjugated nanoparticles were able to specifically bind to the mannose receptor of J774E macrophages (Figure 6A).17
This targeted binding to antigen-presenting cells could broaden the applications of nanoparticle-based vaccines. In the field of oncology, FNP has been utilized to create folic acid-targeted nanoparticles for precise localization to KB tumor cells (Figure 6B).
In collaboration with Janssen Pharmaceutics, Elias et al. demonstrated that scaffold proteins based on a consensus human fibronectin domain, known as Centryrins, can be employed for targeted nanoparticle therapeutics.
EGFR-targeting centryrins were applied to PS-b-PEG nanoparticles and effectively localized to HER2 breast cancer cells (Figure 6C).
Unlike most studies that formulate nanoparticles first and then modify the surface, functionalization can also take place before nanoparticle formation by directly reacting the targeting ligand onto the stabilizing polymer.
Pre-modifying the polymer before FNP enables precise quantification of the functionalization percentage on the nanoparticle surface. Moreover, tuning of the functionalized surfaces is achieved by combining modified polymer with specific proportions of unmodified polymer during FNP.
In an example, LHRH hormone was affixed to the end of a PS-b-PEG polymer and then used to formulate FNP nanoparticles with varying degrees of functionalization.18 In vitro results demonstrated robust nanoparticle uptake by MS578T breast cancer cells (Figure 6D).
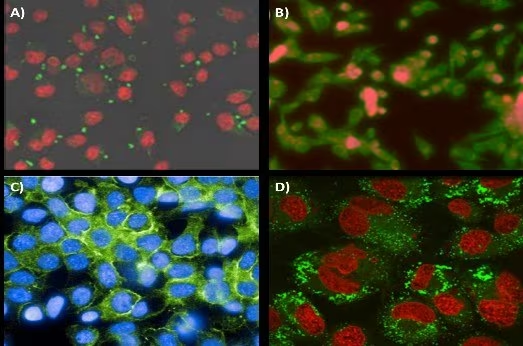
Figure 6. Targeting application of FNP nanoparticles. (A) Mannoside-targeted nanoparticle localization to the mannose receptor of J774E macrophages. Conjugation of mannoside to the PEG outer-corona of the nanoparticles. Nanoparticles are visualized in green, while cells are in red. (B) Folic acid-modified nanoparticles targeting KB tumor line cells. Nanoparticle fluorescence is shown in red, while cells are stained green. (C) Centyrin scaffold targeting GFR receptors on HER2 line cells. (D) Uptake of 80 nm nanoparticles with surface conjugated luteinizing hormone-releasing hormone (LHRH) into MS578T breast cancer cells. Nanoparticles and cells are visualized in green and red, respectively. Image Credit: Merck
Applications in encapsulating water-soluble actives
While FNP effectively encapsulates hydrophobic APIs with a log P >5, it also demonstrates the capability for encapsulating less hydrophobic drugs. Currently, two approaches are under investigation to broaden FNP’s capabilities in encapsulating hydrophilic APIs.
In inverse Flash NanoPrecipitation (iFNP), precipitation of a hydrophilic active occurs using an organic anti-solvent stream. In contrast to a conventional FNP nanoparticle, this “inverse” nanoparticle has hydrophobic ends pointing out and hydrophilic tails pointing inward.
In a subsequent FNP coating step, a hydrophilic polymer is applied to the iFNP nanoparticle to transition it into an aqueous phase (Figure 7A).19 An alternative method for encapsulating hydrophilic APIs involves the use of hydrophobic ion pairing (HIP).
Introducing a counter-ion to electrostatically pair with the hydrophilic API effectively deactivates the charged groups, allowing the resulting ion pair to precipitate during FNP. Gindy et al. applied this method to ion-pair and encapsulate siRNA with a cationic lipid, achieving efficiencies greater than 70% (Figure 7B).21
Ristroph et al. also conducted a review on the encapsulation of peptides and small molecule APIs using HIP.21
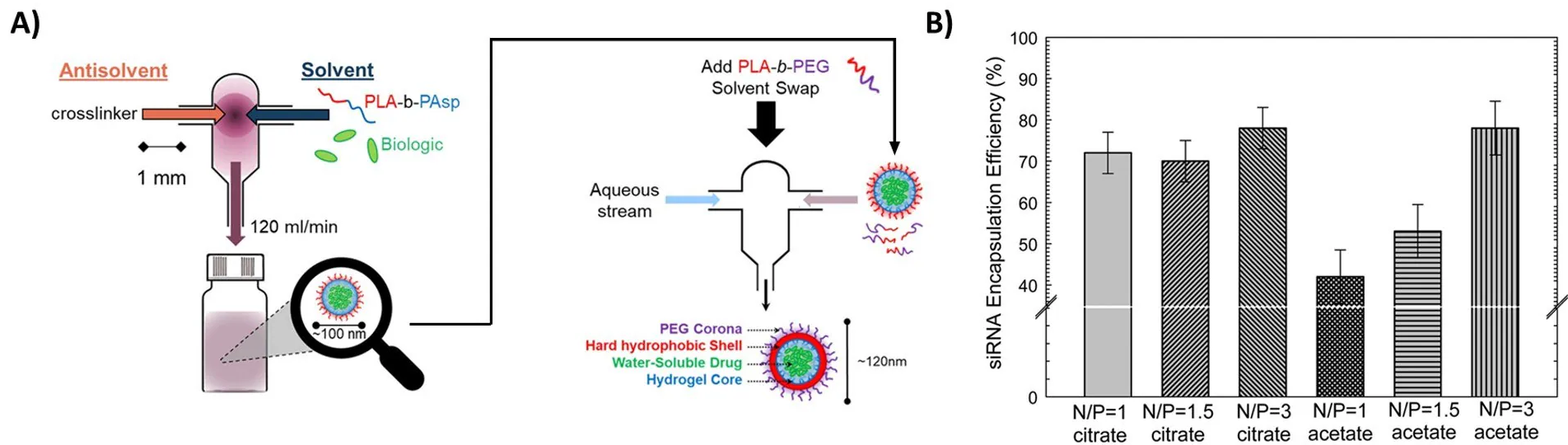
Figure 7. Encapsulation of hydrophilic actives (A) Procedure of iFNP. Precipitation of a biologic or another hydrophilic active occurs by mixing with an organic antisolvent. The resulting inverse nanoparticle is then coated with a second polymer to form a water-dispersible nanoparticle. Reproduced with permission from reference 20, copyright 2014 ACS Publishing. (B) Encapsulation of siRNA using charge pairing. Neutralization of anionic siRNA by cationic lipids allows for the formation of siRNA nanoparticles with very high encapsulation efficiency. Reproduced with permission from reference 21, copyright 2019 Royal Society of Chemistry.
Conclusion
Nanomedicine is dynamic, with ongoing advancements in formulation techniques and therapies. The FNP platform offers a method for producing small-scale laboratory samples for research and has a straightforward pathway to large-scale commercial production.
It accommodates a broad range of actives, from hydrophobic to soluble actives rendered hydrophobic through ion-pairing, as well as soluble oligonucleotides, peptides, and proteins by inverse-FNP.
As a platform technology, FNP is continuously evolving to both broaden applications and enhance the process itself.
This article highlights only a selected array of biomedical applications, aiming to inspire further innovations in nanoparticle-based therapies and diagnostics. A video tutorial demonstrating the operation of CIJ and MIVM mixers for FNP nanoparticle formation has also been published.22
References
- Johnson BK, Prud'homme RK. 2003. Flash NanoPrecipitation of Organic Actives and Block Copolymers using a Confined Impinging Jets Mixer. Aust. J. Chem.. 56(10):1021. https://doi.org/10.1071/ch03115
- Pagels RF, Edelstein J, Tang C, Prud?homme RK. 2018. Controlling and Predicting Nanoparticle Formation by Block Copolymer Directed Rapid Precipitations. Nano Lett.. 18(2):1139-1144. https://doi.org/10.1021/acs.nanolett.7b04674
- Kumar V, Wang L, Riebe M, Tung H, Prud?homme RK. 2009. Formulation and Stability of Itraconazole and Odanacatib Nanoparticles: Governing Physical Parameters. Mol. Pharmaceutics. 6(4):1118-1124. https://doi.org/10.1021/mp900002t
- Liu Y, Cheng C, Liu Y, Prud?homme RK, Fox RO. 2008. Mixing in a multi-inlet vortex mixer (MIVM) for flash nano-precipitation. Chemical Engineering Science. 63(11):2829-2842. https://doi.org/10.1016/j.ces.2007.10.020
- Markwalter CE, Prud'homme RK. 2018. Design of a Small-Scale Multi-Inlet Vortex Mixer for Scalable Nanoparticle Production and Application to the Encapsulation of Biologics by Inverse Flash NanoPrecipitation. Journal of Pharmaceutical Sciences. 107(9):2465-2471. https://doi.org/10.1016/j.xphs.2018.05.003
- Feng J, Markwalter CE, Tian C, Armstrong M, Prud?homme RK. 2019. Translational formulation of nanoparticle therapeutics from laboratory discovery to clinical scale. J Transl Med. 17(1): https://doi.org/10.1186/s12967-019-1945-9
- Suk JS, Xu Q, Kim N, Hanes J, Ensign LM. 2016. PEGylation as a strategy for improving nanoparticle-based drug and gene delivery. Advanced Drug Delivery Reviews. 9928-51. https://doi.org/10.1016/j.addr.2015.09.012
- Pagels RF, Edelstein J, Tang C, Prud?homme RK. 2018. Controlling and Predicting Nanoparticle Formation by Block Copolymer Directed Rapid Precipitations. Nano Lett.. 18(2):1139-1144. https://doi.org/10.1021/acs.nanolett.7b04674
- Gindy ME, Prud'homme RK. 2009. Multifunctional nanoparticles for imaging, delivery and targeting in cancer therapy. Expert Opinion on Drug Delivery. 6(8):865-878. https://doi.org/10.1517/17425240902932908
- Zhang Y, Feng J, McManus SA, Lu HD, Ristroph KD, Cho EJ, Dobrijevic EL, Chan H, Prud?homme RK. 2017. Design and Solidification of Fast-Releasing Clofazimine Nanoparticles for Treatment of Cryptosporidiosis. Mol. Pharmaceutics. 14(10):3480-3488. https://doi.org/10.1021/acs.molpharmaceut.7b00521
- Feng J, Zhang Y, McManus SA, Qian R, Ristroph KD, Ramachandruni H, Gong K, White CE, Rawal A, Prud’homme RK. Amorphous nanoparticles by self-assembly: processing for controlled release of hydrophobic molecules. Soft Matter. 15(11):2400-2410. https://doi.org/10.1039/c8sm02418a
- Pansare VJ, Rawal A, Goodwin A, Beyerinck R, Prud?homme RK, Friesen DT, Grass M, Muske-Dukes A, Vodak DT. 2018. Millisecond Self-Assembly of Stable Nanodispersed Drug Formulations. Mol. Pharmaceutics. 15(2):495-507. https://doi.org/10.1021/acs.molpharmaceut.7b00866
- Lu HD, Lim TL, Javitt S, Heinmiller A, Prud?homme RK. 2017. Assembly of Macrocycle Dye Derivatives into Particles for Fluorescence and Photoacoustic Applications. ACS Comb. Sci.. 19(6):397-406. https://doi.org/10.1021/acscombsci.7b00031
- Lu HD, Wilson BK, Heinmiller A, Faenza B, Hejazi S, Prud?homme RK. 2016. Narrow Absorption NIR Wavelength Organic Nanoparticles Enable Multiplexed Photoacoustic Imaging. ACS Appl. Mater. Interfaces. 8(23):14379-14388. https://doi.org/10.1021/acsami.6b03059
- Pinkerton NM, Gindy ME, CaleroDdelC VL, Wolfson T, Pagels RF, Adler D, Gao D, Li S, Wang R, Zevon M, et al. 2015. Single-Step Assembly of Multimodal Imaging Nanocarriers: MRI and Long-Wavelength Fluorescence Imaging. Adv. Healthcare Mater.. 4(9):1376-1385. https://doi.org/10.1002/adhm.201400766
- Tang C, Edelstein J, Mikitsh JL, Xiao E, Hemphill AH, Pagels R, Chacko A, Prud?homme R. Biodistribution and fate of core-labeled 125I polymeric nanocarriers prepared by Flash NanoPrecipitation (FNP). J. Mater. Chem. B. 4(14):2428-2434. https://doi.org/10.1039/c5tb02172c
- Lu HD, Wang LZ, Wilson BK, McManus SA, Jumai?an J, Padakanti PK, Alavi A, Mach RH, Prud?homme RK. 2018. Copper Loading of Preformed Nanoparticles for PET-Imaging Applications. ACS Appl. Mater. Interfaces. 10(4):3191-3199. https://doi.org/10.1021/acsami.7b07242
- D'Addio SM, Baldassano S, Shi L, Cheung L, Adamson DH, Bruzek M, Anthony JE, Laskin DL, Sinko PJ, Prud'homme RK. 2013. Optimization of cell receptor-specific targeting through multivalent surface decoration of polymeric nanocarriers. Journal of Controlled Release. 168(1):41-49. https://doi.org/10.1016/j.jconrel.2013.02.004
- Akbulut M, Ginart P, Gindy ME, Theriault C, Chin KH, Soboyejo W, Prud'homme RK. 2009. Generic Method of Preparing Multifunctional Fluorescent Nanoparticles Using Flash NanoPrecipitation. Adv. Funct. Mater.. 19(5):718-725. https://doi.org/10.1002/adfm.200801583
- Markwalter CE, Pagels RF, Hejazi AN, Gordon AGR, Thompson AL, Prud?homme RK. 2020. Polymeric Nanocarrier Formulations of Biologics Using Inverse Flash NanoPrecipitation. AAPS J. 22(2): https://doi.org/10.1208/s12248-019-0405-z
- Gindy ME, DiFelice K, Kumar V, Prud?homme RK, Celano R, Haas RM, Smith JS, Boardman D. 2014. Mechanism of Macromolecular Structure Evolution in Self-Assembled Lipid Nanoparticles for siRNA Delivery. Langmuir. 30(16):4613-4622. https://doi.org/10.1021/la500630h
- Ristroph KD, Prud'homme RK. Hydrophobic ion pairing: encapsulating small molecules, peptides, and proteins into nanocarriers. Nanoscale Adv.. 1(11):4207-4237. https://doi.org/10.1039/c9na00308h
- Markwalter CE, Pagels RF, Wilson BK, Ristroph KD, Prud'homme RK. Flash NanoPrecipitation for the Encapsulation of Hydrophobic and Hydrophilic Compounds in Polymeric Nanoparticles. JoVE.(143): https://doi.org/10.3791/58757
About Merck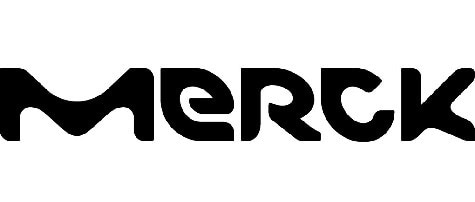
Our pursuit is progress for people everywhere. That's why we take a closer look at things, ask questions, and think ahead.
We've been around for more than 350 years, yet our majority owners are still the descendants of Friedrich Jacob Merck, the man who founded our company in Darmstadt, Germany in 1668.
From advancing gene-editing technologies and discovering unique ways to treat the most challenging diseases to enabling the intelligence of devices – the company is everywhere.
We are Merck. The only exceptions are the United States and Canada. Here we operate as EMD Serono in the Biopharma business, as MilliporeSigma in the Life Science business, and as EMD Performance Materials in the materials business.
Our life science business
We provide infinite solutions to solve the toughest problems in life science in collaboration with the global scientific community. Our tools, services, and digital platforms empower scientists and engineers at every stage, helping deliver breakthrough therapies more quickly.
Focus areas
With our three business units, we are a leading worldwide supplier of tools, high-grade chemicals, and equipment for academic labs, biotech, and biopharmaceutical manufacturers, as well as the industrial sector.
- Research Solutions provides our academic customers with the chemicals and tools needed to make scientific discovery easier and faster.
- Process Solutions provides drug manufacturers with process development expertise and technologies, such as continuous bioprocessing.
- Applied Solutions offers both testing kits and services to ensure that our food is safe to eat and our water is clean to drink.
Sponsored Content Policy: News-Medical.net publishes articles and related content that may be derived from sources where we have existing commercial relationships, provided such content adds value to the core editorial ethos of News-Medical.Net which is to educate and inform site visitors interested in medical research, science, medical devices and treatments.